A synchrotron facility is a laboratory where scientists can examine molecular structures and surfaces in a far more detailed way than before. Researchers in areas such as biology, physics, chemistry, environment, geology, engineering and medicine can utilise this technology.
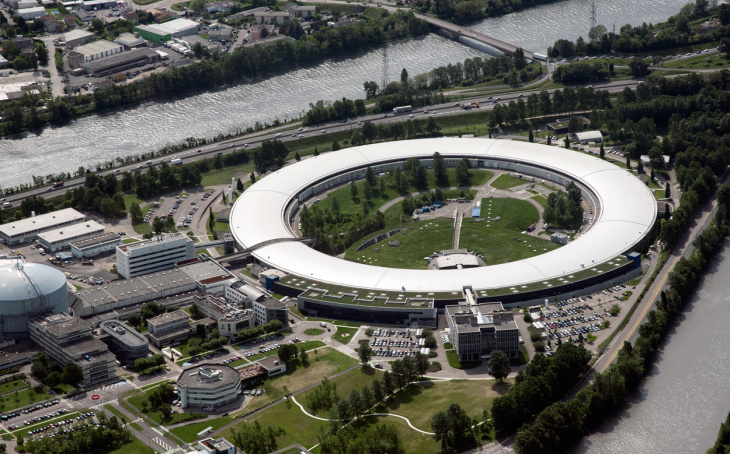
The ESRF light source in Grenoble
© ESRF 2015
A synchrotron is an extremely powerful source of X-rays. It builds on the physical phenomenon that a moving electron emits energy when it changes direction. If the electron is moving fast enough, the emitted energy, called synchrotron radiation, is at X-ray wavelength. Synchrotron radiation is millions of times more brilliant than anything a physician’s X-ray machine can produce. The technology provides opportunities to make new discoveries and products in fields such as materials, medicine and the environment.
Various techniques are used in the experiments: imaging, spectroscopy and scattering. The techniques are often combined and together they offer researchers opportunities to study and develop new drugs, efficient batteries and solar cells, as well as alloys, paper, fabrics and plastics with new functions. Pollutants in water and soil can be measured in order to identify new ways to tackle contamination. Historical and archaeological objects can be examined without damaging them. Healthy and diseased cells and tissue can be analysed as a basis for developing new treatments.
However, the majority of the experiments are for basic research, which aims to give us insights into the workings of the smallest components in materials. This knowledge is necessary for pursuing more applied research such as the examples mentioned above.
European Synchrotrons are organised in the League of European Light Sources (LEAPS).
Learn more about synchrotrons (LEAPS Homepage)
For research purposes, neutrons are released from their role as a component part of matter, and are used as probes with which researchers can look inside a very wide variety of materials. With neutrons one can, for instance, look inside a big car engine, investigate drug delivery, see how plants uptake water, get insights into the development of superconductors, etc..
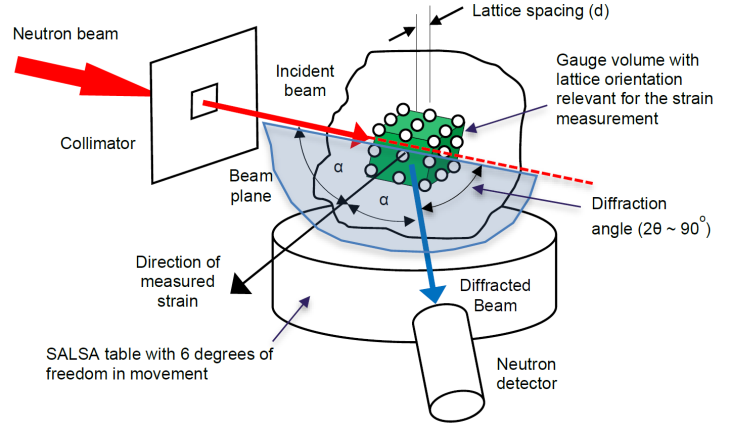
© Matt Roy, U-Man
In a neutron scattering experiment, a neutron beam passes through the sample or technical component under investigation. By observing how the direction or velocity of the neutron changes, researchers learn about the structure, composition or dynamics of the sample on an atomic scale. Having this basic information we can understand the physical, chemical or biological properties of a material.
When beams of neutrons are used to probe small samples of materials they have the power to reveal what cannot be seen using other types of radiation. Neutrons appear to behave either as particles or as waves or as microscopic magnetic dipoles.
It is these specific properties which enable them to yield information which is often impossible to obtain using other techniques.
Beams of neutrons can be scattered by materials. If the neutron energy is chosen correctly, then their wavelength is similar to the distances between atoms or molecules, and the pattern of scattered neutrons can be used to produce an image of the atomic structure.
What can neutrons show?
Neutrons can show us the structure of matter, where atoms are located and how they move. Neutrons can also help to analyse the magnetic properties of materials, at the atomic scale. Neutrons show hydrogen atoms and can be used to highlight special structural features.
How are neutrons produced?
Together with protons, neutrons form the nucleus of most atoms. Neutrons are therefore part of all the matter that surrounds us. To be used as a scientific probe and produce a beam they have to be released from the nucleus by a process called fission, as happens in a nuclear reactor using Uranium or by firing a high-energy beam of protons into a neutron-rich element such as lead or tungsten – a process called spallation. We thus need a Large Scale Facility to produce neutrons for science, like e.g. some of the partners of EASI-STRESS.
© https://www.sine2020.eu
League of European Neutron Sources (LENS)
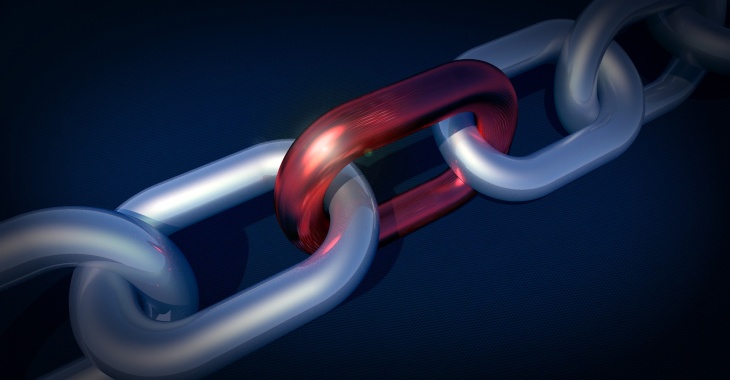
© Pixabay
Residual stresses are mechanical stresses in a body, which is not experiencing external forces and which is in thermal equilibrium. They are caused by plastic deformation or thermal processes, such as welding or rapid cooling (quenching) after heating.
To make assumptions on the strength and lifetime of a component, assessing of residual stresses important. The reason is the superposition principle, which means that in a first approximation a linear superposition (addition) of applied load during operation and residual stress distributions represent the total stress distribution within a workpiece or a component. Residual stresses have influence on many properties of a component, such as strength, fatigue behaviour, corrosion resistance and others. Failure of workpiece in most cases occurs due to plastic deformation or fracture when subjected to tensile loads. Here, e.g., because of notch effects, the near-surface area is critical. This means that compressive residual stresses in the near-surface zone can be beneficial with respect to workpiece or component lifetime. In some industrially produced components, such as generator shafts, or pressure vessels, terms of delivery often include specifications regarding maximum residual stress values and permissible residual stress distributions [1, 2].
[1] Hauk, V.: Structural and Residual Stress Analysis by Nondestructive Methods, Elsevier, 1997
[2] Reimers, W.; Pyzalla, A. R.; Schreyer, A.; Clemens, H.: Neutrons and Synchrotron Radiation in Engineering Materials Science, Viley VCH, 2008, S. 115 ff.
About residual stress